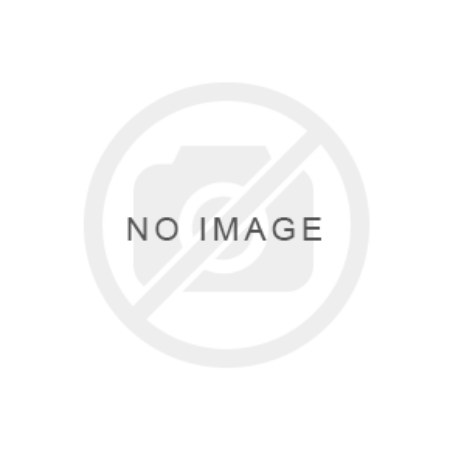
Friday, December 27, 2024 The Tibet-Nepal Railway project, part of China’s Belt and Road Initiative, aims to connect Kerung in southern Tibet to Kathmandu, Nepal. Set to cost approximately 38 billion yuan (USD 5.5 billion), it faces significant challenges due to the difficult Himalayan terrain. A feasibility study funded by China will determine the optimal route and railway type. While the project promises to enhance regional connectivity and economic growth, it raises environmental and geopolitical concerns, particularly regarding its impact on the fragile ecosystem and relations with neighboring India. Successful completion will rely on advanced engineering and careful environmental management.